Pigtailed Photodiodes
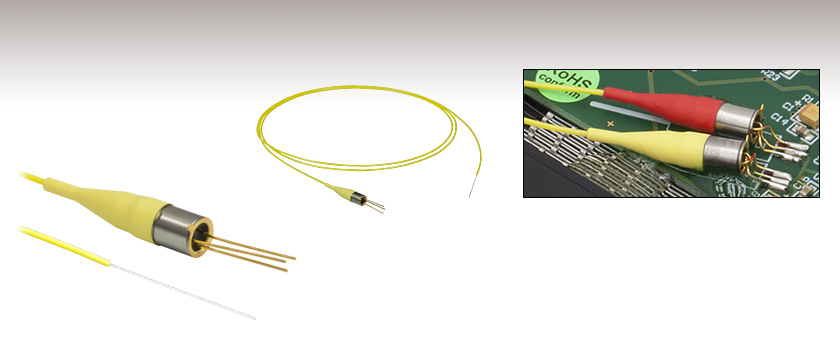
- High-Speed Photodiode, Coupling Lens, and Fiber
in One Easy-to-Use Package - Si PIN Photodiode
- Reinforced Fiber
Photodiode
FDSP Pigtailed
Photodiode
SM or MM Fiber
A Pigtailed Photodiode Soldered to the Circuit Board Inside
the INT-MZI-850 Fiber-Based Interferometer
Application Idea

Please Wait
Mounted and Unmounted Detectors |
---|
Unmounted Photodiodes (200 - 2600 nm) |
Calibrated Photodiodes (350 - 1800 nm) |
Mounted Photodiodes (200 - 1800 nm) |
Thermopile Detectors (0.2 - 15 µm) |
Photovoltaic Detectors (2.0 - 10.6 µm) |
Pigtailed Photodiodes (320 - 1000 nm) |
Features
- Multimode Version for 320 - 1000 nm
- Single Mode Versions for 610 - 770 nm and 780 - 970 nm
- Ideal for Applications Requiring High-Speed Signal Detection
- Low Bias Voltage
Thorlabs' Pigtailed Photodiodes are high-speed, fiber pigtailed, Si PIN photodiodes designed for light detection in the visible to near infrared range. These photodiodes are particularly suitable for measurement of pulsed or CW fiber-coupled light sources by converting the optical power into an electric current. They provide low dark current and terminal capacitance with increased bias voltabe making them ideal for use in optical communications, high-speed photometry, and monitoring applications.
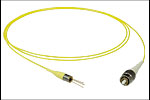
Click to Enlarge
A Pigtailed Photodiode terminated with an SM FC/PC connector. Custom termination of the fiber end of our pigtailed photodiodes is available upon request.
The FDSP Series Pigtailed Photodiodes are offered with a single or multimode fiber, actively aligned for optimum coupling efficiency, sensitivity, and stability. The FDSP625 uses a graded-index multimode fiber suitable for use with wavelengths between 320 and 1000 nm. FDSP660 and FDSP780 use single mode fibers, which suppress mode interferences (also called MPI - multi path interference), an essential property for signal detection in fiber-based interferometers. They are designed for low back reflection and are specified for use at 610 - 770 nm and 780 - 970 nm, respectively. Please see the info icons below for a detailed list of fiber and detector properties.
The housing is a stainless steel bushing used for active coupling of the fiber to the photodiode. The fiber is reinforced with a Ø900 µm loose tube outer jacket and a rubber boot, which relieves fiber bending stresses.
Custom Connectorization
To complement our pigtailed photodiodes, we have a wide range of fiber connectors (single mode and multimode) and a fiber termination kit. Custom fiber termination with industry-standard fiber connectors is available upon request. Contact our Tech Support for more information. Please note that the PDs sold below are not calibrated, and specifications may differ slightly from lot to lot. We also offer calibrated photodiodes, which come with with NIST-traceable calibration. Our photodiodes can be reverse voltage biased using the PBM42 DC Bias Module for higher optical power detection; for more information on voltage biasing as well as the noise floor, please see the Lab Facts tab.
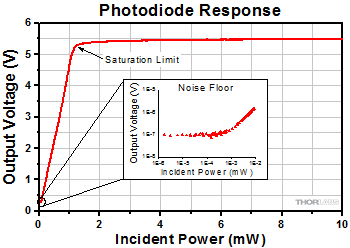
Click to Enlarge
Figure 1. Overview of the Photodiode's Response Curve,
Highlighting the Saturation Limit and the Noise Floor
Thorlabs Lab Fact: Photodiode Saturation Limit and Noise Floor
We present laboratory measurements of the saturation limit and noise floor of a Thorlabs silicon photodiode. While all photodiodes function similarly, there are a number of parameters that affect the noise floor and saturation limit of a photodiode including the sensor temperature, resistivity, reverse bias voltage, responsivity, and system bandwidth. Here we investigated the effect of reverse bias voltage and load resistance within a silicon-based photodiode detection system. Increasing the reverse bias increased the saturation limit and had minimal effect on the noise floor. Decreasing the load resistance decreased the noise floor until reaching the noise of the measurement system, but also decreased the saturation limit. These results demonstrate some of the considerations necessary for choosing the reverse bias voltage and load resistance, and emphasize that noise sources within all of the components must be considered when creating a detection system.
For our experiment we used the FDS100 Si Photodiode as the photodiode under investigation. The collimated output of a fiber-pigtailed laser diode was used as the light source with output power from 0 to 50 mW. The collimated beam was incident upon a beamsplitter that transmitted the majority of the light to the photodiode under investigation and reflected the rest towards a reference power sensor. The photodiode response was then evaluated under various resistive loads and with different reverse bias voltages.
The plots to the right and below summarize the measured results for the various tested configurations. From these graphs the changes to the photodiode's linear response, noise floor, and saturation limit can be observed under different reverse voltage biases and load resistances. Figure 1 provides an overview of the photodiode response with a reverse voltage bias of 5 V and resistive load of 10 kΩ. The photodiode saturated at the upper limit of the response when the output photovoltage approached the reverse bias voltage. The noise floor at the lower limit of the response was a result of dark current and the thermal noise from the resistive load (Johnson noise). Figure 2 summarizes the results obtained using the photodiode with a 1 kΩ resistive load and various reverse bias voltages. It illustrates that the saturation limit can be raised by increasing the reverse bias voltage (within specification). Figure 3 summarizes the results from using the photodiode with a 5 V reverse bias voltage and various resistive loads. It illustrates that the slope of the photovoltage response increased as the load resistance was increased. Figure 4 summarizes the noise floor results obtained using a 0 V reverse bias voltage and various resistive loads. The noise floor increased when larger load resistances were used. It is important to note that the 1 kΩ data was measured above the theoretical Johnson noise due to the voltage noise within the measurement system. Minimal change in the overall noise floor was seen when using a 5 V reverse bias voltage. For details on the experimental setup employed and these summarized results, please click here.
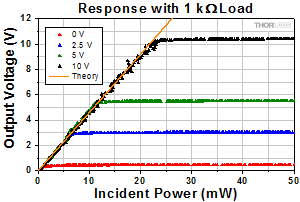
Click to Enlarge
Figure 2. Photovoltage Dependence on Reverse Bias Voltage
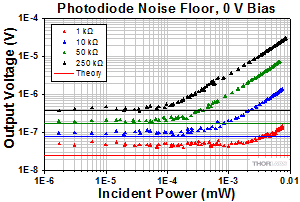
Click to Enlarge
Figure 4. Noise Floor with Various Resistive Loads
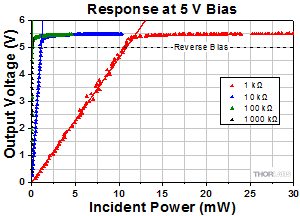
Click to Enlarge
Figure 3. Response with Various Resistive Loads
Photodiode Tutorial
Theory of Operation
A junction photodiode is an intrinsic device that behaves similarly to an ordinary signal diode, but it generates a photocurrent when light is absorbed in the depleted region of the junction semiconductor. A photodiode is a fast, highly linear device that exhibits high quantum efficiency and may be used in a variety of different applications.
It is necessary to be able to correctly determine the level of the output current to expect and the responsivity based upon the incident light. Depicted in Figure 1 is a junction photodiode model with basic discrete components to help visualize the main characteristics and gain a better understanding of the operation of Thorlabs' photodiodes.
Figure 1: Photodiode Model
Photodiode Terminology
Responsivity
The responsivity of a photodiode can be defined as a ratio of generated photocurrent (IPD) to the incident light power (P) at a given wavelength:
Modes of Operation (Photoconductive vs. Photovoltaic)
A photodiode can be operated in one of two modes: photoconductive (reverse bias) or photovoltaic (zero-bias). Mode selection depends upon the application's speed requirements and the amount of tolerable dark current (leakage current).
Photoconductive
In photoconductive mode, an external reverse bias is applied, which is the basis for our DET series detectors. The current measured through the circuit indicates illumination of the device; the measured output current is linearly proportional to the input optical power. Applying a reverse bias increases the width of the depletion junction producing an increased responsivity with a decrease in junction capacitance and produces a very linear response. Operating under these conditions does tend to produce a larger dark current, but this can be limited based upon the photodiode material. (Note: Our DET detectors are reverse biased and cannot be operated under a forward bias.)
Photovoltaic
In photovoltaic mode the photodiode is zero biased. The flow of current out of the device is restricted and a voltage builds up. This mode of operation exploits the photovoltaic effect, which is the basis for solar cells. The amount of dark current is kept at a minimum when operating in photovoltaic mode.
Dark Current
Dark current is leakage current that flows when a bias voltage is applied to a photodiode. When operating in a photoconductive mode, there tends to be a higher dark current that varies directly with temperature. Dark current approximately doubles for every 10 °C increase in temperature, and shunt resistance tends to double for every 6 °C rise. Of course, applying a higher bias will decrease the junction capacitance but will increase the amount of dark current present.
The dark current present is also affected by the photodiode material and the size of the active area. Silicon devices generally produce low dark current compared to germanium devices which have high dark currents. The table below lists several photodiode materials and their relative dark currents, speeds, sensitivity, and costs.
Material | Dark Current | Speed | Spectral Range | Cost |
---|---|---|---|---|
Silicon (Si) | Low | High Speed | Visible to NIR | Low |
Germanium (Ge) | High | Low Speed | NIR | Low |
Gallium Phosphide (GaP) | Low | High Speed | UV to Visible | Moderate |
Indium Gallium Arsenide (InGaAs) | Low | High Speed | NIR | Moderate |
Indium Arsenide Antimonide (InAsSb) | High | Low Speed | NIR to MIR | High |
Extended Range Indium Gallium Arsenide (InGaAs) | High | High Speed | NIR | High |
Mercury Cadmium Telluride (MCT, HgCdTe) | High | Low Speed | NIR to MIR | High |
Junction Capacitance
Junction capacitance (Cj) is an important property of a photodiode as this can have a profound impact on the photodiode's bandwidth and response. It should be noted that larger diode areas encompass a greater junction volume with increased charge capacity. In a reverse bias application, the depletion width of the junction is increased, thus effectively reducing the junction capacitance and increasing the response speed.
Bandwidth and Response
A load resistor will react with the photodetector junction capacitance to limit the bandwidth. For best frequency response, a 50 Ω terminator should be used in conjunction with a 50 Ω coaxial cable. The bandwidth (fBW) and the rise time response (tr) can be approximated using the junction capacitance (Cj) and the load resistance (RLOAD):
Noise Equivalent Power
The noise equivalent power (NEP) is the input signal power that results in a signal-to-noise ratio (SNR) of 1 in a 1 Hz output bandwidth. This is useful, as the NEP determines the ability of the detector to detect low level light. In general, the NEP increases with the active area of the detector and is given by the following equation:
Here, S/N is the Signal to Noise Ratio, Δf is the Noise Bandwidth, and Incident Energy has units of W/cm2. For more information on NEP, please see Thorlabs' Noise Equivalent Power White Paper.
Terminating Resistance
A load resistance is used to convert the generated photocurrent into a voltage (VOUT) for viewing on an oscilloscope:
Depending on the type of the photodiode, load resistance can affect the response speed. For maximum bandwidth, we recommend using a 50 Ω coaxial cable with a 50 Ω terminating resistor at the opposite end of the cable. This will minimize ringing by matching the cable with its characteristic impedance. If bandwidth is not important, you may increase the amount of voltage for a given light level by increasing RLOAD. In an unmatched termination, the length of the coaxial cable can have a profound impact on the response, so it is recommended to keep the cable as short as possible.
Shunt Resistance
Shunt resistance represents the resistance of the zero-biased photodiode junction. An ideal photodiode will have an infinite shunt resistance, but actual values may range from the order of ten Ω to thousands of MΩ and is dependent on the photodiode material. For example, and InGaAs detector has a shunt resistance on the order of 10 MΩ while a Ge detector is in the kΩ range. This can significantly impact the noise current on the photodiode. For most applications, however, the high resistance produces little effect and can be ignored.
Series Resistance
Series resistance is the resistance of the semiconductor material, and this low resistance can generally be ignored. The series resistance arises from the contacts and the wire bonds of the photodiode and is used to mainly determine the linearity of the photodiode under zero bias conditions.
Common Operating Circuits
Figure 2: Reverse-Biased Circuit (DET Series Detectors)
The DET series detectors are modeled with the circuit depicted above. The detector is reverse biased to produce a linear response to the applied input light. The amount of photocurrent generated is based upon the incident light and wavelength and can be viewed on an oscilloscope by attaching a load resistance on the output. The function of the RC filter is to filter any high-frequency noise from the input supply that may contribute to a noisy output.
Figure 3: Amplified Detector Circuit
One can also use a photodetector with an amplifier for the purpose of achieving high gain. The user can choose whether to operate in Photovoltaic of Photoconductive modes. There are a few benefits of choosing this active circuit:
- Photovoltaic mode: The circuit is held at zero volts across the photodiode, since point A is held at the same potential as point B by the operational amplifier. This eliminates the possibility of dark current.
- Photoconductive mode: The photodiode is reversed biased, thus improving the bandwidth while lowering the junction capacitance. The gain of the detector is dependent on the feedback element (Rf). The bandwidth of the detector can be calculated using the following:
where GBP is the amplifier gain bandwidth product and CD is the sum of the junction capacitance and amplifier capacitance.
Effects of Chopping Frequency
The photoconductor signal will remain constant up to the time constant response limit. Many detectors, including PbS, PbSe, HgCdTe (MCT), and InAsSb, have a typical 1/f noise spectrum (i.e., the noise decreases as chopping frequency increases), which has a profound impact on the time constant at lower frequencies.
The detector will exhibit lower responsivity at lower chopping frequencies. Frequency response and detectivity are maximized for
Pulsed Laser Emission: Power and Energy Calculations
Determining whether emission from a pulsed laser is compatible with a device or application can require referencing parameters that are not supplied by the laser's manufacturer. When this is the case, the necessary parameters can typically be calculated from the available information. Calculating peak pulse power, average power, pulse energy, and related parameters can be necessary to achieve desired outcomes including:
- Protecting biological samples from harm.
- Measuring the pulsed laser emission without damaging photodetectors and other sensors.
- Exciting fluorescence and non-linear effects in materials.
Pulsed laser radiation parameters are illustrated in Figure 1 and described in the table. For quick reference, a list of equations is provided below. The document available for download provides this information, as well as an introduction to pulsed laser emission, an overview of relationships among the different parameters, and guidance for applying the calculations.
Equations: |
||||
![]() |
and | ![]() |
||
![]() |
||||
![]() |
||||
![]() |
||||
Peak power and average power calculated from each other: |
||||
![]() |
and | ![]() |
||
Peak power calculated from average power and duty cycle*: | ||||
![]() |
*Duty cycle (![]() |
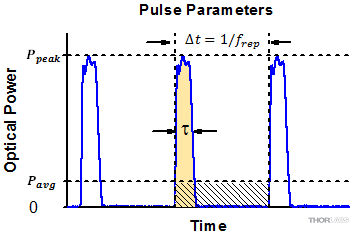
Click to Enlarge
Figure 1: Parameters used to describe pulsed laser emission are indicated in the plot (above) and described in the table (below). Pulse energy (E) is the shaded area under the pulse curve. Pulse energy is, equivalently, the area of the diagonally hashed region.
Parameter | Symbol | Units | Description | ||
---|---|---|---|---|---|
Pulse Energy | E | Joules [J] | A measure of one pulse's total emission, which is the only light emitted by the laser over the entire period. The pulse energy equals the shaded area, which is equivalent to the area covered by diagonal hash marks. | ||
Period | Δt | Seconds [s] | The amount of time between the start of one pulse and the start of the next. | ||
Average Power | Pavg | Watts [W] | The height on the optical power axis, if the energy emitted by the pulse were uniformly spread over the entire period. | ||
Instantaneous Power | P | Watts [W] | The optical power at a single, specific point in time. | ||
Peak Power | Ppeak | Watts [W] | The maximum instantaneous optical power output by the laser. | ||
Pulse Width | ![]() |
Seconds [s] | A measure of the time between the beginning and end of the pulse, typically based on the full width half maximum (FWHM) of the pulse shape. Also called pulse duration. | ||
Repetition Rate | frep | Hertz [Hz] | The frequency with which pulses are emitted. Equal to the reciprocal of the period. |
Example Calculation:
Is it safe to use a detector with a specified maximum peak optical input power of 75 mW to measure the following pulsed laser emission?
- Average Power: 1 mW
- Repetition Rate: 85 MHz
- Pulse Width: 10 fs
The energy per pulse:
seems low, but the peak pulse power is:
It is not safe to use the detector to measure this pulsed laser emission, since the peak power of the pulses is >5 orders of magnitude higher than the detector's maximum peak optical input power.
Posted Comments: | |
Pierrick Cheiney
 (posted 2020-10-27 04:16:16.83) Dear Sir/Madam,
Could you give info on the typical polarization dependant losses ?
Best regards,
Pierrick Cheiney dpossin
 (posted 2020-10-28 06:24:04.0) Dear Pierrick,
Thank you for your feedback. Unfortunately we do not have data on the PDL. michel lintz
 (posted 2020-07-27 04:20:02.483) Hi,
You say these photodiodes (FDSP780 for instance) are designed for low back-reflection. But you do not give a number. Could you give a typical value? Thanks dpossin
 (posted 2020-07-28 08:59:46.0) Dear Michael,
Thank you for your feedback! Because the minimum detectable power depends on the wavelength you are using as well as the output voltage depends on the load resistance you apply in the circuit we can not really give numbers on that which are valid in general. Here are some considerations according to the detection limit for you:
Using the NEP the minium output can be calculated by P_min=NEP_min*Res_max/Res(lambda)*sqrt(BW).
The used symbols are:
BW: electrical bandwidth
P_min: minimum detectable optical power
NEP_min: NEP at the responsivity maximum
Res_max: responsivity maximum
Res(lambda): responsivity at lambda
Please also look up here: https://www.thorlabs.com/images/TabImages/Noise_Equivalent_Power_White_Paper.pdf for further information.
Also the minium detectable power depend on the sensitivity of your scope. The output voltage can be assumed the following:
V_out=(I_D+Res(lambda)*P_o)*R_L
The used symbols are:
V_out: output voltage
I_D: dark current
P_o: optical input power
R_L: load resistance
Please see here: https://www.thorlabs.com/images/TabImages/Photodetector_Lab.pdf for reference. melanie Holmes
 (posted 2020-05-18 14:46:24.82) Is it possible to use these photodiodes with your benchtop photodiode amplifier ? wskopalik
 (posted 2020-05-25 04:24:27.0) This is a response from Wolfgang at Thorlabs. Thank you very much for your inquiry!
Yes, these photodiodes can also be used with the benchtop photodiode amplifier PDA200C. You would just need to make a custom cable to connect the photodiodes to the BNC input port of the amplifier.
I will contact you directly to provide further assistance. Roger Holten
 (posted 2020-01-25 08:51:25.667) It would be nice to see a version of this using an InGAs detector for better longer wavelength performance and flatter performance in the 1020-1080nm range MKiess
 (posted 2020-01-28 05:46:19.0) This is a response from Michael at Thorlabs. Thank you very much for your inquiry. We have the possibility to manufacture Pigtailed InGAs photodiodes as custom versions. I have contacted you directly to discuss details. CChang
 (posted 2016-06-09 15:03:08.587) Dear Thorlab
I am looking for the pigtailed detector for 1590 nm. It is expected to have FC/PC connector, SMF-28 fiber and detector integrated into one piece.
Do you have extra information or relevent vendor that you can forward it to me. Thank you very much.
Chih-Hsuan Chang
CChang@spectrasensors.com besembeson
 (posted 2016-06-09 03:17:58.0) Response from Bweh at Thorlabs USA: I will contact you regarding this please. c.kimsoon
 (posted 2016-05-11 22:31:41.54) Hi, my name is Kim Soon. I found this product is suitable for me in developing my prototype. However, those product do not have the specific wavelength that I required. May I know is it posibble to customize it? I need a wavelength between 500~530nm.Thanks. shallwig
 (posted 2016-05-12 04:28:00.0) This is a response from Stefan at Thorlabs. Thank you very much for your inquiry. The FDSP625 is specified for 320-1000nm. I will contact you directly to discuss your application in detail. eloktionov
 (posted 2015-08-11 18:02:57.43) Hello,
Can't get any signal from FDSP625. Built a circuit for reverse bias as in tutorial. Connecting to 50 Ohm oscilloscope input (1mV/dev). Use 300mW@405 nm CW laser diode as a light source. Have checked contacts and bias voltage (+7 V) reaching pin 1. But still can't get any signal. Pinned respectively to DET10A internal board in parallel. Still no result. Am I missing something? shallwig
 (posted 2015-08-12 10:47:07.0) This is a response from Stefan at Thorlabs. Thank you very much for your inquiry. I have contacted you directly to troubleshoot this problem in detail. |

Item # | Info | Detector Type | Rise/Fall Timea | NEP (WHz-1\2) | Dark Current | Terminal Capacitance | Wavelength Range | Fiber | Core Diameter | NA | Compatible Sockets |
---|---|---|---|---|---|---|---|---|---|---|---|
FDSP625 | ![]() |
Silicon/PIN | 700 ps (Typical) | 3.1 x 10-15 | 0.01 nA Typ. 0.5 nA Max |
3 pF | 320 - 1000 nm | Graded-Index, Multimode | 62.5 µm | 0.27 | STO46S STO46P |
FDSP660 | ![]() |
700 ps | 610 - 770 nm | Nufern 630-HP, Single Mode | 4 µm | 0.13 | |||||
FDSP780 | ![]() |
700 ps | 780 - 970 nm | Nufern 780-HP, Single Mode | 5 µm |